Menu
Publications
2025
2024
2023
2022
2021
2020
2019
2018
2017
2016
2015
2014
2013
2012
2011
2010
2009
2008
2007
2006
2005
2004
2003
2002
2001
Editor-in-Chief
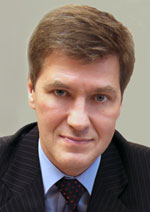
Nikiforov
Vladimir O.
D.Sc., Prof.
Partners
doi: 10.17586/2226-1494-2021-21-6-837-847
Vulnerabilities in the quantum key distribution system induced under a pulsed laser attack
Read the full article

Article in Russian
For citation:
Abstract
For citation:
Ruzhitskaya D.D., Zhluktova I.V., Petrov M.A., Zaitsev K.A., Acheva P.P., Zunikov N.A., Shilko A.V., Aktas D., Johlinger F., Trefilov D.O., Ponosova A.A., Kamynin V.A., Makarov V.V. Vulnerabilities in the quantum key distribution system induced under a pulsed laser attack. Scientific and Technical Journal of Information Technologies, Mechanics and Optics, 2021, vol. 21, no. 6, pp. 837–847 (in Russian). doi: 10.17586/2226-1494-2021-21-6-837-847
Abstract
Quantum communication protocols are considered secure provided that all devices included in the system are fully characterized, and side channels are closed. However, as a result of laser radiation exposure, it is possible to change quantum communication systems components’ characteristics. This leads to vulnerabilities appearing in the quantum key distribution system. In this paper, we consider the effect of pulsed laser radiation on fiber-optic isolators used in quantum communication systems. Isolators protect the source of the system from attacking optical radiation coming from the “eavesdropping” side via the quantum channel. Lowering the isolation factor can bring the entire system out of a secure state. This gives an eavesdropper access to information about the secret key. The scenario of the most probable attack to the source of the quantum key distribution system via a pulsed laser was simulated. The experimental setup provided exposure of fiber isolators with pulsed laser radiation at a wavelength of 1064 nm (within the transparency window of the isolators) with a mean power up to 840 mW in four different pulse generation modes. The isolation factor and throughput of tested samples were monitored using a laser diode with a wavelength of 1550 nm and average power of 10.5 mW. Spectrally selective splitters were used to separate the lasers. It is shown that the isolation factor (isolator attenuation in the direction from the quantum channel to the system) at a wavelength of 1550 nm decreases from the initial value of 59.1 dB to 24.5 dB. The throughput (in the direction from the system to the quantum channel) at the same wavelength decreases from 0.6 dB to 1.2–12.3 dB or remains the same, depending on the acting pulsed laser radiation parameters. Temperature monitoring showed that the temperature of the isolator body changes insignificantly when exposed to pulsed radiation. The obtained effects of changing the isolation coefficient and throughput can be explained by the presence of nonlinear effects in the magneto-optical crystal of the isolator. The results of the work can be applicable in the practical evaluation of quantum communication systems security, in particular, the security evaluation of quantum key distribution systems. The results can be used to prepare the standards for certification procedures for assessing the security of quantum communication systems. The work gives recommendations for enhancing signal source unit security in quantum communication systems. Namely, as countermeasures to protect against the effects of pulsed laser radiation, it is proposed to set optical fuses with a limited threshold power, detectors for monitoring input optical radiation power, and narrow-band optical filters at the entrance of the quantum communication system.
Keywords: quantum communication, quantum cryptography, quantum communication systems vulnerabilities, laser-damage attack, pulsed lasers
Acknowledgements. This work is funded by the Ministry of Science and Higher Education of the Russian Federation, Agreement No. 075-11-2021-078 dated September 29, 2021.
References
Acknowledgements. This work is funded by the Ministry of Science and Higher Education of the Russian Federation, Agreement No. 075-11-2021-078 dated September 29, 2021.
References
-
Bennett C.H., Brassard G. Quantum cryptography: Public key distribution and coin tossing. Theoretical Computer Science, 2014, vol. 560, part 1, pp. 7–11. https://doi.org/10.1016/j.tcs.2014.05.025
-
Scarani V., Bechmann-Pasquinucci H., Cerf N.J., Dušek M., Lütkenhaus N., Peev M. The security of practical quantum key distribution. Reviews of Modern Physics, 2009, vol. 81, no. 3, pp. 1301–1350. https://doi.org/10.1103/RevModPhys.81.1301
-
Lo H.-K., Curty M., Tamaki K. Secure quantum key distribution. Nature Photonics, 2014, vol. 8, no. 8, pp. 595–604. https://doi.org/10.1038/nphoton.2014.149
-
Wiechers C., Lydersen L., Wittmann C., Elser D., Skaar J., Marquardt C., Makarov V., Leuchs G. After-gate attack on a quantum cryptosystem. New Journal of Physics, 2011, vol. 13, no. 1, pp. 013043. https://doi.org/10.1088/1367-2630/13/1/013043
-
Bugge A.N., Sauge S., Ghazali A.M.M., Skaar J., Lydersen L., Makarov V. Laser damage helps the eavesdropper in quantum cryptography. Physical Review Letters, 2014, vol. 112, no. 7, pp. 070503. https://doi.org/10.1103/PhysRevLett.112.070503
-
Pang X.-L., Yang A.-L., Zhang C.-N., Dou J.-P., Li H., Gao J., Jin X.-M. Hacking quantum key distribution via injection locking. Physical Review Applied, 2020, vol. 13, no. 3, pp. 034008. https://doi.org/10.1103/PhysRevApplied.13.034008
-
Sun S.-H., Xu F., Jiang M.-S., Ma X.-C., Lo H.-K., Liang L.-M. Effect of source tampering in the security of quantum cryptography. Physical Review A, 2015, vol. 92, no. 2, pp. 022304. https://doi.org/10.1103/PhysRevA.92.022304
-
Jain N., Anisimova E., Khan I., Makarov V., Marquardt C., Leuchs G. Trojan-horse attacks threaten the security of practical quantum cryptography. New Journal of Physics, 2014, vol. 16, no. 12, pp. 123030. https://doi.org/10.1088/1367-2630/16/12/123030
-
Huang A., Navarrete Á., Sun S.-H., Chaiwongkhot P., Curty M., Makarov V. Laser-seeding attack in quantum key distribution. Physical Review Applied, 2019, vol. 12, no. 6, pp. 064043. https://doi.org/10.1103/PhysRevApplied.12.064043
-
Lucamarini M., Choi I., Ward M.B., Dynes J.F., Yuan Z.L., Shields A.J. Practical security bounds against the trojan-horse attack in quantum key distribution. Physical Review X, 2015, vol. 5, no. 3, pp. 031030. https://doi.org/10.1103/PhysRevX.5.031030
-
Tan H., Li W., Zhang L., Wei K., Xu F. Chip-based quantum key distribution against trojan-horse attack. Physical Review Applied, 2021, vol. 15, no. 6, pp. 064038. https://doi.org/10.1103/PhysRevApplied.15.064038
-
Makarov V., Bourgoin J.-P., Chaiwongkhot P., Gagné M., Jennewein T., Kaiser S., Kashyap R., Legré M., Minshull C., Sajeed S. Creation of backdoors in quantum communications via laser damage. Physical Review A, 2016, vol. 94, no. 3, pp. 030302. https://doi.org/10.1103/PhysRevA.94.030302
-
Huang A., Li R., Egorov V., Tchouragoulov S., Kumar K., Makarov V. Laser-damage attack against optical attenuators in quantum key distribution. Physical Review Applied, 2020, vol. 13, no. 3, pp. 034017. https://doi.org/10.1103/PhysRevApplied.13.034017
-
Ponosova A., Ruzhitskaya D., Chaiwongkhot P., Egorov V., Makarov V., Huang A. Isolation reduction of quantum cryptography systems induced by continuous-wave high-power laser. Available at: http://qutes.org/wp-content/uploads/2020/02/PonosovaAA.pdf (accessed: 20.10.2021).
-
Ruzhitskaya D., Ponosova A., Jöhlinger F., Chaiwongkhot P., Egorov V., Aktas D., Rarity J.G., Erven C., Makarov V., Huang A. Protecting QKD sources against light-injection attacks. Available at: http://www.vad1.com/publications/ruzhitskaya2020.QCrypt-subm43.pdf (accessed: 20.10.2021).
-
Wood R.M. Laser-Induced Damage of Optical Materials. CRC Press, 2003, 241 p.
-
Vojna D., Slezăk O., Lucianetti A., Mocek T. Verdet constant of magneto-active materials developed for high-power Faraday devices. Applied Sciences, 2019, vol. 9, no. 15, pp. 3160.app9153160
-
Hui R., O’Sullivan M. Fiber Optic Measurement Techniques. Academic Press, 2009, 672 p. https://doi.org/10.3390/
-
Smith A.V., Do B.T. Bulk and surface laser damage of silica by picosecond and nanosecond pulses at 1064 nm. Applied Optics, 2008, vol. 47, no. 26, pp. 4812–4832. https://doi.org/10.1364/AO.47.004812
-
Hadley G.R. High-power pulse propagation in optical fibers. OFC/NFOEC 2008 - 2008 Conference on Optical Fiber Communication/National Fiber Optic Engineers Conference, 2008, pp. 4528567. https://doi.org/10.1109/OFC.2008.4528567
-
Lamaignère L., Gaudfrin K., Donval T., Natoli J., Sajer J.-M., Penninckx D., Courchinoux R., Diaz R. Laser-induced damage of fused silica optics at 355 nm due to backward stimulated Brillouin scattering: experimental and theoretical results. Optics Express, 2018, vol. 26, no. 9, pp. 11744–11755. https://doi.org/10.1364/OE.26.011744
-
Smith A.V., Do B.T., Hadley G.R., Farrow R.L. Optical damage limits to pulse energy from fibers. IEEE Journal of Selected Topics in Quantum Electronics, 2009, vol. 15, no. 1, pp. 153–159. https://doi.org/10.1109/JSTQE.2008.2010331
-
Zhluktova I.V., Filatova S.A., Trikshev A.I., Kamynin V.A., Tsvetkov V.B. All-fiber 1125 nm spectrally selected subnanosecond source. Applied Optics, 2020, vol. 59, no. 29, pp. 9081–9086. https://doi.org/10.1364/AO.401668